Home > Medical Research Archives > Issue 149 > Timing in Morphogenesis of the Developing Nervous System: Relation to Genetic Programming and Exogenous Teratogenesis
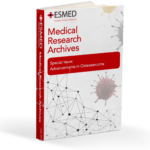
Published in the Medical Research Archives
Jun 2023 Issue
Timing in Morphogenesis of the Developing Nervous System: Relation to Genetic Programming and Exogenous Teratogenesis
Published on Jun 26, 2023
DOI
Abstract
Timing is essential to morphogenesis of the embryonic and fetal nervous system and intricately associated with onset of expression of developmental genes. Mitotic cell cycles are timed. Teratogenic toxins and other adverse influences are teratogenic in the context of timing by interfering with developmental processes. Each of the 3 axes of the neural tube is associated with two opposing genetic gradients. Many neural malformations can be analyzed pathologically as interference with genetic gradients of one or more of the axes, even if the specific genetic mutation is not yet identified. Examples of cerebral malformations closely associated with defective timing are agenesis of forebrain commissures (anterior and hippocampal commissures form 3 weeks before corpus callosum), neuronogenesis and gliogenesis in disorders of the mTOR signaling pathway (time of expression of postmitotic somatic mutation in relation to the 33 mitotic cycles of neuroepithelium determines extent of lesion of focal cortical dysplasia II or hemimegalencephaly), prosencephalic cleavage and eversion (holoprosencephaly; telencephalic flexure for Sylvian fissure), neuromeric disorders of segmentation of the neural tube (deletion of neuromeres; Chiari I), maturation of individual neurons, synaptogenesis (precocious synaptic circuitry in holoprosencephaly; delayed synapse formation in many genetic/metabolic encephalopathies), myelination (delay in many congenital encephalopathies) and neural crest migrations including craniofacial development (facial dysmorphisms in many genetic syndromes and hypertelorism in some cases of callosal agenesis). Timing of relation of genes in cascade or inhibitory genes acting on others is a key element of normal and abnormal morphogenesis.
Author info
Introduction
Timing is the essence of embryonic and fetal morphogenesis, above all in the nervous system. The moment of onset of expression of an essential developmental gene and sometimes its effect on a cascade of downstream genes, including inhibitory effects at times, affects development in a unique manner. Not only spontaneous genetic mutations but induced effects on genetic expression from fetal exposure to exogenous toxins, drugs including alcohol, radiation and other adverse influences explains teratogenesis and resulting cerebral malformations related to timing of physiological morphogenesis. Effects on the nervous system, whether intrinsic or extrinsic, also can affect extra- neural development through abnormal neural crest formation or migration. An example of the latter is craniofacial development and an embryological explanation of the pathogenesis of facial dysmorphisms. Timing is primordial in evolution: circadian clocks are self-sustained biological oscillators that are found even in the most primitive prokaryotic and eukaryotic organisms 1. They are used in more evolved life forms for many types of temporal oscillating circuits in the brain ranging from central cardiac and respiratory control to periodic “pacemaker” thalamocortical discharges in the fetus to sleep cycles to menstrual periods. Timed circadian rhythms are molecular clocks composed of transcriptional-translational feedback loops 2-4. Such clocks may be entrained by photoreceptors 4 or by isoforms of transcription factors such as CDC20 that inhibit the anaphase of mitosis to induce prolonged cell cycle arrest 5. Acceleration of mitotic cell cycles in the brain may lead to neoplasia whereas extending or arresting cell cycles may result in neural fewer cells generated and hypoplasia of cerebral structures.
The following examples in this review are offered to highlight the importance of timing in neural development, to explain many cerebral malformations and to provide correlation between genetic expression and morphogenesis.
Timing in neural tube formation
Each of the 3 axes of the neural tube is associated with two opposing genetic gradients. The longitudinal axis has rostro-caudal and caudo- rostral gradients; the vertical axis has dorso-ventral and ventro-dorsal gradients; the horizontal axis has medio-lateral and latero-medial gradients. These genetic gradients led to the fundamental morphological form of all animals exhibiting bilateral symmetry, as a rostral or head end and tail or caudal end, dorsal and ventral surfaces and axial and lateral body structures including extremities. Early gene regulatory programmes explain larval to adult metamorphoses in simple animals such as flatworms (planaria) and segmented worms (annelids) 6,7. The same genetic and timing principles that apply to the body as a whole apply also to the nervous system.
Many neural malformations can be analyzed pathologically as interference with genetic gradients of one or more of the axes, even if the specific genetic mutation is not identified. Holoprosencephaly is a prototype malformations that exhibits disturbance in all three axes of the neural tube: in the longitudinal axis, the rostro- caudal gradient of genetic expression is defective so that forebrain, diencephalic and midbrain structures are more dysplastic than hindbrain and spinal cord; the dorso-ventral gradient explains why the dorsal tectum of the midbrain (collicular plate) may be a continuous dome-shaped midline uncleaved structure and the cerebral aqueduct is atretic but the ventrally located cerebral peduncles are distinct and paired as normal; the mediolateral gradient presents non-cleavage of the prosencephalon and more severely dysplastic cortex in the medial than in the more lateral aspects of the forebrain 8-11. These genetic gradients of the 3 axes are precisely timed and coordinated with each other.
Rhombencephalosynapsis of the cerebellum appears as agenesis of the vermis and more dysplastic cerebellar cortex in medial than in lateral aspects of the cerebellar hemispheres, whereas in ponto-cerebellar hypoplasias the latero-medial gradient is affected so that the vermis may be almost normal, paravermal cerebellar cortex is mildly dysplastic and lateral cerebellar cortex is severely dysplastic 11.
Timing of neural crest
Progenitor neural crest cells form at the lateral margins of the neural placode before the latter bends to create the neural tube. These cells are carried to the dorsal surface by neural fold bending where they meet in the dorsal midline as the neural tube closes and almost immediately begin to migrate peripherally to form not only neural tissues such as autonomic ganglion cells and Schwann cells, but also many other non-neural cells and tissues, including melanocytes, adipocytes and the Purkinje conduction system of the cardiac septum. Differentiation of neural crest cells occurs only after their migration is completed and they are in their mature sites in the body. This cellular maturation is precisely timed in coordination with differentiation of the surrounding tissues, whether visceral organs, heart, gastrointestinal tract or skin.
Prosencephalic and especially mesencephalic neural crest tissue forms most of the craniofacial structures, including the cartilages and membranous bones of the face (but not endochondral bone of the cranial base), orbits and most of the cranial vault (except occipital bones), connective tissues, most of the globe of the eye (except retina, choroid and iris), peripheral nerve sheaths, dura mater and leptomeninges. Facial dysmorphisms in various genetic syndromes and chromosomopathies are the result of faulty timing of neural crest migration.
Timing in mitotic cycles and disorders of the mtor signaling pathway
The mammalian target of rapamycin (mTOR) is a cascade of genetic pathways essential to cellular lineage, differentiation and maturation 12,13. It begins by regulating microtubules, which form the fibres of the mitotic spindle and subsequently the growth and morphogenesis of the differentiating cell, neural cells (neurons and glial cells) in particular. In the central nervous system, the best documented examples of disorders of the mTOR pathway are tuberous sclerosis complex (TSC), hemimegalencephaly (HME) and focal cortical dysplasia type II (FCD II). In each of these post- zygotic somatic mutations involve some, but not all, progenitor cells so that some clones of cells are normal and others are megalocytic, dysplastic neurons and glial cells with altered or deficient functions such as synaptogenesis.
Timing is the determinant factor distinguishing FCD II and HME, which are really the same disease neuropathologically and genetically 12-14. In the human, approximately 33 mitotic cycles of the periventricular neuroepithelium are needed to produce all neurons of the cerebral cortex and subcortical telencephalic nuclei, because of exponential logarithmic increase in numbers of neurons with each mitotic cycle 15. Rodents require only 10-11 mitotic cycles for their simpler cortex with fewer neurons and no convolutions. If the post- zygotic somatic mutation is initiated in a late stage of the 33 cycles (e.g cycle 31), a small dysplastic cortical lesion is created by the migratory neuroblasts that might involve only a single gyrus in the mature brain as FCD II. An earlier cycle (e.g. cycle 22) produces a more extensive lesion involving several adjacent gyri. An even earlier cycle (e.g. cycle 14) causes involvement of the entire cerebral hemisphere as HME and the earliest lesions (e.g. cycle 5) may involve the whole neuroepithelium on one side that extends caudally to cause hemihypertrophy of a lateral half of the brainstem and cerebellum as well, known as total HME. It is timing of the expression of the somatic mutation, therefore, that determines the extent of the dysplastic lesion in mTOR disorders. TSC is more complex genetically than HME/FCD II because it involves germline mutations of the TSC1 or TSC2 gene in addition to the post-zygotic somatic mutations 16.
One factor that is critical to the termination of mitotic cycles is the differentiation of an ependyma at the ventricular surface. The ventricles initially are bordered by neuroepithelium with most of the mitotic activity at the margin of the ventricle. Ependyma differentiates in a precise time-linked sequence, first as a pseudostratified epithelium and only later as a simple cuboidal epithelium. The lateral ventricles do not complete their ependymal lining until 22 weeks gestation 17. After the ependyma forms in a region of the ventricles, neuroepithelial cells are post-mitotic pre-migratory neuroblasts or glioblasts. After 16 weeks few new neuroblasts differentiate; the great majority of cells are of glial lineage.
Prosencephalic cleavage and eversion
At 4-5 weeks post-conceptional age, the single midline prosencephalon of the forebrain at the rostral end of the neural tube undergoes sagittal cleavage by formation of the interhemispheric fissure, to create paired telencephalic hemispheres. In mammals including humans the dorsal part of the forebrain then grows medially to form telencephalic hemispheres enclosing lateral ventricles within the brain, called inversion of the hemispheres. In teleostean (bony) fishes, however, the dorsal part grows laterally rather than medially as eversion of the hemispheres; rather than a lateral ventricle surrounded by the forebrain, the ventricular equivalent is at the surface of the cerebral hemispheres with cerebrospinal fluid in the space between the surface ependyma and the cerebral tissue beneath 18.
The prototype human cerebral malformation that is a disorder of prosencephalic cleavage and inversion is alobar and semilobar holoprosencephaly 19,20, associated with many other dysgeneses of the brain and a similar phenotype with a variable genotype of at least 14 different mutations, some associated with trisomy-13. Timing is a key factor in the pathogenesis of this severe developmental disorder of the brain. An insult or initial expression of a mutation that occurs at the precise time of cleavage impairs this process. Holoprosencephaly also is a good example of a disorder involving all 3 axes of the neural tube with defective genetic gradients in each 9.
Timing in neuromeric segmentation of the neural tube
After closure of the posterior and anterior neuropores of the neural tube, the tube becomes segmented with segmental compartments called neuromeres 21-30. More specific neuroembryological terminology are rhombomeres of the hindbrain (future brainstem), mesomeres of the midbrain and prosomeres of the forebrain (future diencephalon and telecephalon). The future spinal cord was traditionally thought to be non-segmented and the paired nerve roots and associated blood vessels appearing segmented because of segmentation of the underlying somites that periodically grouped emerging roots, though it also has been long known that the parasympathetic nerves emerge from the cervical and sacral portions and sympathetic chain ganglia are from the thoraco-lumbar portions of the spinal cord. It is only recently recognized that the spinal cord also is a true segmented structure with different genes expressed in some segments and not in others 8,25-30. Similarly, the original 1910 concept of Herrick that the prosomeres were merely a rostral continuation of the hindbrain rhombomeres 31, which he reiterated in 1948 32 was challenged by Rubenstein 33, Puelles et al. 34-36, and by Carstens and Sarnat 30 so that concepts of forebrain development have undergone major revision. Anatomical demarcation of various future structures of the embryonic and early fetal forebrain is indicated by the course of radial glial fibres 37. Timing is a major factor in understanding neural tube segmentation at all levels.
The hindbrain was the initial part of the brain studied with molecular genetic correlations 26-31. The most important developmental gene families involved in rhombencephalic segmentation are the homeobox (HOX), engrailed (EN1, EN2), paired homeobox (PAX), wingless (WNT) genes. One of the purposes of segmentation is to limit the longitudinal migration of neurons so that brainstem nuclei composed of neurons of similar type can form. The barriers between compartments or rhombomeres involve a transverse glial membrane, web of neurofilament-containing cellular processes and decreased mitoses at levels between rhombomeres, but the barrier to cellular migration is more chemical than physical. The precise localization of rhombomeric genetic expressions and their timing was established before other parts of the neural tube were so defined. Disorders of time-linked segmentation of the hindbrain or other parts of the neural tube and can result in deletions of entire neuromeres 38-43.
Timing in the midline crossing of commissural axons
Agenesis of the corpus callosum is the most frequent cerebral malformation of liveborn infants. The most frequent dysgenesis of all is anencephaly, but involved embryos and fetuses rarely survive prenatally or live only a few seconds or minutes after delivery if liveborn. The three major forebrain commissures are the corpus callosum, anterior commissure and hippocampal commissure or psalterium. The diagnosis of callosal agenesis may be made prenatally by ultrasound or by fetal MRI after 18 weeks gestation. The neurons or origin of the axons of the corpus callosum are the small pyramidal neurons of layer 3 of the cerebral cortex, though they are preceded by transitory pioneer axons from the subplate zone 44. Layer 3 neurons are well preserved in number and morphology without degenerative changes or apoptosis in agenesis of the corpus callosum and their axons that cannot cross the midline are diverted to aberrant pathways, such as the bundle of Probst which is large posterior growing fascicle on the mediolateral aspect of both cerebral hemisphere that displaces laterally and straightens the shape of the lateral ventricle. Less frequent aberrant projections of callosal axons enter the posterior limb of the internal capsule to accompany the corticospinal and corticobulbar tracts, and aberrant crossings of individual axons at other sites of the telencephalic hemispheres that are below the resolution of current imaging techniques 44. Agenesis of the corpus callosum may occur as an isolated cerebral dysgenesis or may be part of a more complex malformation, chromosomopathy or genetic syndrome. Commissures interconnect homologous structures on the two sides of the neuraxis, whereas in decussations axons also traverse the midline but the origin and destination of these fibres are different and usually at a different level in the longitudinal axis of the neural tube.
In normal cerebral morphogenesis, the first pioneer axons of the corpus callosum cross the midline at about 10 weeks gestation, but the anterior commissure and hippocampal commissure form earlier at 7 weeks; the corpus callosum achieves its mature morphology of its various parts by 115 days 45-47. It is useful, therefore, when viewing the MRI of infants or children with agenesis of the corpus callosum to specifically look also for the anterior commissure; coronal views demonstrated both structures well in the same plane. If the corpus callosum is absent and the anterior commissure present (sometimes hypertrophic because it also contains some aberrant callosal axons), one may infer that the onset of genetic expression or the epigenetic teratogenic influence occurred between 7 and 10 weeks gestation. If both the corpus callosum and the anterior commissure are absent, the pathogenetic insult occurred before 7 weeks gestation 11,44. In partial agenesis of the corpus callosum, further refinement of timing can sometimes be made because normal axonal passage begins in the splenium, earlier than in the genu or rostrum.
The pathogenesis of agenesis of the corpus callosum and anterior commissure also is time-linked. Callosal axons pass through the dorsal part of the lamina terminalis, a transitory embryonic and early fetal structure that forms the rostral wall of the 3rd ventricle and demarcates the telencephalon from diencephalon; anterior commissural axons pass through a somewhat more ventral position in the middle of the lamina terminalis 48. A primitive glial wall exists at the lamina terminalis that must undergo physiological apoptosis to form a bridge for commissural axons to cross the midline. This bridge forms 1-2 days before the first axons traverse. If there is a genetic mutation that does not enable this critical focal apoptosis, callosal axons meet a “brick wall” rather than a bridge at the midline and are prevented from crossing 48,49.
Timing in maturation of individual neurons
Nervous system development is usually thought of as tissue architecture or morphogenesis. However, the maturation of individual neurons also must be considered as precisely timed simultaneous events that must be temporally coordinated for proper neuronal function 50-53. Neuronal maturation from a neuroblast involves much more than assuming the mature morphology of the type of neuron. Initial axonal sprouting precedes the initial growth of dendrites; intermediate filaments to stiffen and preserve the shapes of neurites must form. Some filaments are immature and transitory (e.g. nestin; vimentin) before being co-expressed and eventually replaced by mature filaments (e.g. neurofilaments). The proteins to form microfilaments must first be synthesized before these molecules can be assembled as filaments. Axonal trajectories to nearby or distant synaptic sites are largely guided by extracellular molecules, most of which are secreted by glial cells, for example keratan sulfate which also insulates axonal fascicles and keeps them pure with axons of the same origin using the same transmitter and contacting target neurons at a similar destination, rather than a mixture of axons of different types 54. Some types of neurons never develop axons and form dendro-dendritic synapses; the highest density of these unique neurons is in the thalamus and the olfactory bulb. Some neurons never develop any neurites, either axons or dendrites, and secrete transmitter chemicals into the bloodstream, for example chromaffin cells of the adrenal medulla and carotid body.
Many aspects of neuronal maturation are not so easily observed microscopically. The development of polarity of the plasma membrane and maintenance of a resting membrane potential requires a preceding energy supply system: Na+/K+ adenosine triphosphatase (ATPase) pump and mitochondrial proliferation for energy and to enable biosynthesis of a chemical substance that serves as a neurotransmitter. Other cellular organelles also must form to fabricate the neurotransmitter and transport it by axoplasmic flow. Plasma membranes must form ion channels and receptors of transmitters. All closely integrated cellular processes of neuronal maturation are precisely timed and genetically programmed, as are the morphology of specific neuronal types and the type of transmitters they synthesize. The expression of neuronal proteins also is precisely timed, some appearing late as the neuron is nearly mature (e.g. neuronal nuclear antigen or NeuN) and others appearing much sooner as the neuroblast differentiates from the primitive neuroepithelial cell (e.g. microtubule-associated proteins; calretinin; parvalbumin) 53,54. Genetic disorders of the mTOR pathway are good examples of cellular dysplasia of both neurons and glial cells 11-13.
Timing of Synaptogenesis
Synaptogenesis is a late step in neuronal maturation that enable the formation of local synaptic circuits and more widespread networks, without which the nervous system could not function. Synapse formation requires an important glial component as well, including for uptake of excessive transmitter secreted into synaptic clefts 55,56. In every part of the immature brain there is a precise and constant timing of appearance of synapses which can be demonstrated in sections of fetal brain by immunoreactivity to substances that form synaptic vesicles, regardless of the neurotransmitter contained within, and are constant patterns at any given gestational age 57-61. In some metabolic encephalopathies of prenatal life synaptogenesis may be delayed. In other conditions, such as holoprosencephaly, there may be precocious synaptic formation in the cortex and retina, out of synchrony with other cellular maturational processes and conducive to early epileptic circuitry expressed as seizures in the early postnatal period 62,63.
Timing of myelination
Another late step in maturation is myelination of axons, which also is precisely timed and predictable in all white matter tracts of the central nervous system 64-68. Apart from oligodendrocytes, microglia also are important regulators of myelin growth and an integral part of timing of myelination 68,69. Some pathways, such as many intrinsic projections within the brainstem, myelinate within a couple of weeks during fetal life. Long tracts and commissures often require months or years to complete their myelination cycles. The corpus callosum shows initial myelination of axons at about 2 months postnatally by histological stains of tissue sections and at 3-4 months by MRI detection; it does not complete myelination until late adolescence. The corticospinal tract begins myelination during the late 3rd trimester of gestation and is not complete until 2 years of age. The last fascicle to myelinate in the human brain is the ipsilateral frontotemporal association bundle, at age 32 years 64. The subcortical U-fibres have a different anatomy and function from deep white matter and generally myelinate later than deep white matter axons 70. In many malformations and congenital metabolic encephalopathies, myelination is delayed and the sequence may be altered. In some brainstem structures, such as the nucleus/tractus solitarius, delayed synaptogenesis and myelination may be expressed clinically as central hypoventilation with neonatal apnoea or respiratory insufficiency 71.
Conclusions
Gradients of genetic expression along the 3 axes of the neural tube can explain many malformations of the brain even if the precise genetic mutation is unknown. The timing of onset of genetic expression or of exposure to a teratogenic toxin during embryonic and fetal development is a major determinant factor in the type, location and extent of a dysgenesis of the brain. Mitotic cellular cycles also are timed. Timing and morphogenesis are therefore intimately linked.
Ethics Committee Approval: This study was approved by the Conjoint Health Research Ethics Committee of the University of Calgary and Alberta Health Services.
Funding: Alberta Childrens Hospital Research Institute, Grant #60-28450, Project 10027949.
Disclosures: The author has no financial disclosures or conflicts of interest to declare.
In our laboratories, fetal and postnatal autopsy tissues are always treated with respect.
References
1. Pitsawong W, Padua RAP, Grant T, Hoemberger M, Otten R, Bradshaw N, et al. From primordial clocks to circadian oscillators. Nature 2023;616:183-189.
2. Crane BR, Young MW. Interactive features of proteins composing eukaryotic circadian clocks. Ann Rev Biochem 2014;83:191-219.
3. Takashashi TS. Transcriptional architecture of the mammalian circadian clock. Nat Rev Genet 2017;18:164-179.
4. Lin C, Feng S, DeOliveira CC, Crane BR. Cryptochrome-timeless structure reveals circadian clock timing mechanisms. Nature 2023;617:194-199.
5. Tsang M-J, Cheeseman IM. Alternative CDC20 translational isoforms tune mitotic arrest duration. Nature 2023;617:154-161.
6. Pagán OR. The First Brain. The Neuroscience of Planarians. NY, Oxford: Oxford University Press. 2014.
7. Martin-Zamora FM, Liang Y, Guynes K, Carrillo-Baltodano AM, Davies BE, Donnellan RD, et al. Annelid functional geonomics reveal the origins of bilaterian life cycles. Nature 2023;615:105-110.
8. Sarnat HB, Menkes JH. The new neuroembryology: How to construct a neural tube. J cc
9. Sarnat HB. The new neuroembryology: Molecular genetic classification of central nervous system malformations. J Child Neurol 2000;21:675-687.
10. Sarnat HB, Flores-Sarnat L. Morphogenesis timing of genetically-programmed brain malformations in relation to epilepsy. Progr Brain Res 2014;213:181-198.
11. Sarnat HB. The 2016 Bernard Sachs Lecture: Timing in morphogenesis and genetic gradients during normal development and in malformations of the nervous system. Pediatr Neurol 2018;83:3-13.
12. MÜHlebner A, Bongaarts A, Sarnat HB, Scholl T, Aronica E. New insights into a spectrum of developmental malformations related to mTOR dyregulations: Challenges and perspectives. J Anat 2019;235:521-542.
13. Sarnat HB, BLÜmcke I, Miyata H, Vinters HV. Epilepsy: A Comprehensive Textbook. 3rd ed. Chapter 13: Neuropathology of Developmental Disorders Associated with Epilepsy. Philadelphia: Walters Kluwers. 2023. in press.
14. D’Gama AM, Geng Y, Couto JA, Martin B, Boyle EA, LaCoursière CM, et al. mTOR pathway mutations cause hemimegalencephaly and focal cortical dysplasia. Ann Neurol 2015;77:720-725.
15. Caviness VS Jr, Pinto-Lord MC, Evrard P. The development of laminated patterns in the mammalian neocortex. In: Morphogenesis of Pattern Formation. Connelly TG, ed. NY: Raven Press. 1981. pp 103-126.
16. Curatolo P, Specchio N, Aronica E. Advances in the genetics and neuropathology of tuberous sclerosis complex: edging closer to targeted therapy. Lancet Neurol 2022;21(9):843-856.
17. Sarnat HB. Regional differentiation of the human fetal ependyma: immunocytochemical markers. J Neuropathol Exp Neurol 1992;51:58-75.
18. Sarnat HB, Netsky MG. Evolution of the Nervous System. Oxford, NY: Oxford University Press. 1978. pp 324-326.
19. Golden JA. Holoprosencephaly: a defect in brain patterning. J Neuropathol Exp Neurol 1998;57:991-999.
20. Sarnat HB, Flores-Sarnat L. Neuropathological research strategies in holoprosencephaly. J Child Neurol 2001;16:918-931.
21. Kallén B. Early morphogenesis and pattern formation in the central nervous system. In: DeHann (ed). Organogensis. New York: Holt, Rhinehart & Winston, 1965, pp. 107-128.
22. McClure CFW. The segmentation of the primitive vertebrate brain. J Morphol 1980;4:35-56. 103.
23. Lumsden A. The cellular basis of segmentation in the developing hindbrain. Trends Neurosci 1990;13:329-335.
24. Keynes R, Lumsden A. Segmentation and the origin of regional diversity in the vertebrate central nervous system. Neuron 1990;2:1-9.
25. McGinnis W, Krumlauf R. Homeobox genes and axial patterning. Cell. 1992;68:283-302.
26. Keynes R, Krumlauf R, Hox genes and regionalization of the nervous system. Annu Rev Neurosci 1994;17:109-132.
27. Krumlauf R. Hox genes in vertebrate development. Cell 1994;78 :191-201.
28. Joyner AL. Engrailed, Wnt and Pax genes regulate midbrain-hindbrain development. Trends Genet 1996;12:15-20.
29. Joyner AL. Engrailed, Wnt and Pax genes regulate midbrain-hindbrain development. Trends Genet 1997;124:2923-2934.
30. Carstens MH, Sarnat HB. Neuromeres: The timing and sequence of their appearance and their relationship to brain development. In: Developmental Principles of Head and Neck Anatomy: The Neuromeric Basis of Craniofacial Structure. Carstens MH, ed. Berlin, NY: Springer-Verlag. 2023; in press.
31. Herrick CJ. The morphology of the forebrain in amphibia and reptiles. J Comp Neurol Psychol. 1910;20:413-547.
32. Herrick CJ. The Brain of the Tiger Salamander, Amblystoma tigrinum. Chicago: University of Chicago Press. 1948.
33. Rubenstein JLR. The prosomeric model: a proposal for the organization of the embryonic forebrain. Science 1994; 266:578-580.
34. Puelles l, Rubenstein JLR. Expression patterns of homeobox and other putative regulatory genes in the embryonic mouse forebrain suggest a neuromeric pattern. Trends Neurosci 1993; 16:472-479.
35. Puelles L, Rubenstein JLR. Forebrain gene expression domains and the evolving prosomeric model. Trends Neurosci 2003;26:469-476.
36. Puelles L, Harrison M, Paxinos G, Watson C. A developmental ontology for the mammalian brain based on the prosomeric model. Trends Neurosci 2013;36:570-578.
37. Mai JK, Andressen C, Ashwell KWS. Demarcation of prosencephalic regions by CD15-positive radial glia. Eur J Neurosci 1998;10:756-751.
38. Kuemerle B, Zanjani H, Joyner A, et al. Pattern deformities and cell loss in Engrailed-2 mutant mice suggest two separate patterning events during cerebellar development. J Neurosci 1997;17:7881-7889.
39. McMahon AP, Joyner AL, Bradley A, et al. The midbrain-hindbrain phenotype of Wnt- 1−/Wnt-1 mice results from stepwise deletion of engrailed-expressing cells by 9.5 days postcoitum. Cell 1992;69:581-595.
40. Sarnat HB, Benjamin DR, Kletter GB, Seibert JR, Cheyette SR. Agenesis of the mesencephalon and metencephalon with cerebellar hypoplasia: putative mutation of the EN2 gene. Report of 2 cases in early infancy. Pediatr Dev Pathol 2002;5:54-68.
41. Ten Donkelaar HJ, Lammens M, Cruysberg JRM, et al. Development and developmental disorders of the brainstem. In: Ten Donkelaar HJ, Lammens M, Hori A, eds. Clinical Embryology: Development and Developmental Disorders of the Human Central Nervous System. Berlin: Springer-Verlag. 2007
42. Selim LA, Zaki MS, Hussein HA, Saleem SN, Kotoury AS, Issa MY. Developmental abnormalities in mid and hindbrain: a study of 23 Egyptian patients. Egypt J Med Hum Genet 2008;9:215-236.
43. Sarnat HB. Disorders of segmentation of the neural tube: Agenesis of neuromeres. Handb Clin Neurol 2008;87:105-113.
44. Sarnat HB. Embryology and malformation of the forebrain commissures. Handb Clin Neurol 2008;87:67-87.
45. Rakic P, Yakovlev P. Development of the corpus callosum. J Comp Neurol 1968;132:45-72.
46. Loeser JD, Alvord EC Jr. Agenesis of the corpus callosum. Brain 1968;91:553-570.
47. Barr MS, Corbellis MC. The role of the anterior commissure in callosal agenesis. Neuropsychology 2002;16:459-471.
48. Sarnat HB. Transitory and vestigial structures of the developing human nervous system. Pediatr Neurol 2021;123:86-101.
49. Zaki W. Le processus dégénératif au cours du développement du corps calleux. Arch Anat Micr Morphol Exper 1985;74:133-149.
50. Sasaki A, Hirato J, Nakazato Y, Ishida Y. Immunohistochemical study of the early human fetal brain. Acta Neuropathol 1988;76:128- 134.
51. Honig LS, Herrmann K, Shatz CJ. Developmental changes revealed by immunohistochemical markers in human cerebral cortex. Cerebr Cortex 1996;6:794-806.
52. Sarnat HB. Clinical Neuropathology Practice Guide 5-2013: Markers of neuronal maturation. Clin Neuropathol 2013:32:340- 369.
53. Sarnat HB. Immunocytochemical markers of neuronal maturation in human diagnostic neuropathology. Cell Tiss Res 2015;359:279- 294.
54. Sarnat HB. Proteoglycan (keratan sulfate) barrier in developing human forebrain isolates cortical epileptic networks from deep heterotopia, insulates axonal fascicles and explains why axosomatic synapses are inhibitory. J Neuropathol Exp Neurol 2019;78:1147-1159.
55. Allen NJ, Eroglu C. Cell biology of astrocyte- synapse interactions. Neuron 2017;96:697- 708.
56. Sarnat HB, Rao VTS. Glial Cell Pathology in Genetic and Epigenetic Disorders of the Central Nervous System. Handb Clin Neurol 2023; in press.
57. Sarnat HB, Flores-Sarnat L, Trevenen CL. Synaptophysin immunoreactivity in the human hippocampus and neocortex from 6 to 41 weeks of gestation. Journal of Neuropathology and Experimental Neurology 2010;69:234- 245.
58. Sarnat HB, Auer RN, Flores-Sarnat L. Synaptogenesis in the fetal corpus striatum, globus pallidus and substantia nigra. Correlations with striosomes of Graybiel and dyskinesias in premature infants. Journal of Child Neurology 2013;28:60-69.
59. Sarnat HB, Flores-Sarnat L, Auer RN. Sequence of synaptogenesis in the human fetal and neonatal cerebellar system. Part 1. Guillain- Mollaret triangle (dentato-rubro-olivary- cerebellar circuit. Developmental Neuroscience 2013;35:69-81.
60. Sarnat HB, Flores-Sarnat L, Auer RN. Sequence of synaptogenesis in the human fetal and neonatal cerebellar system. Part 2. Pontine nuclei and cerebellar cortex. Developmental Neuroscience 2013;35:317-325.
61. Sarnat HB, Yu W. Maturation and dysgenesis of the human olfactory bulb. Brain Pathol 2016;26:301-318.
62. Sarnat HB, Flores-Sarnat L. Precocious and delayed neocortical synaptogenesis in fœtal holoprosencephaly. Clinical Neuropathology. 2013;32:255-268.
63. Sarnat HB, Resch L, Flores-Sarnat L, Yu W. Precocious synapses in 13.5-week fetal holoprosencephalic cortex and cyclopean retina. Brain and Development 2014;36:463- 471.
64. Yakovlev PI, Lecours A-R. The myelination cycles of regional maturation of the brain. In: Minkowsky A, editor. Regional Development of the Brain in Early Life. Philadelphia: FA Davis, 1967. pp 3-70.
65. Rorke LB, Riggs HE. Myelination in the Brain of the Newborn. Philadelphia, Toronto: Lippincott, 1969.
66. Gilles FH. Myelination in the neonatal brain. Brain Pathol 1976;7:244-8.
67. Kinney HC, Brody BA, Kloman AS, et al. Sequence of central nervous system myelination in human infancy. II. Patterns of myelination in autopsied infants. J Neuropathol Exp Neurol 1988;47(3):217-34.
68. Hughes EG, Appel B. The cell biology of CNS myelination. Curr Opin Neurobiol 2016;39:93- 100.
69. McNamara NB, Munro DAD, Bestard-Cuche N, Uyeda A, Bogle JFJ, Hoffmann A, et al. Microglia regulate central nervous system myelin growth and integrity. Nature 2023;613:120-129.
70. Sarnat HB, Hader W, Flores-Sarnat L, Bello- Espinosa L. Synaptic plexi of the U-fibre layer beneath focal cortical dysplasias: role in epileptic networks. Clin Neuropathol 2018;37:262-276.
71. Sarnat HB, Flores-Sarnat L. Synaptogenesis and myelination in the nucleus/tractus solitarius. Potential role in apnoea of prematurity, congenital central hypoventilation and sudden infant death syndrome. J Child Neurol 2016;31:722-732.
Author Area
Have an article to submit?
Submission Guidelines
Submit a manuscript
Become a member